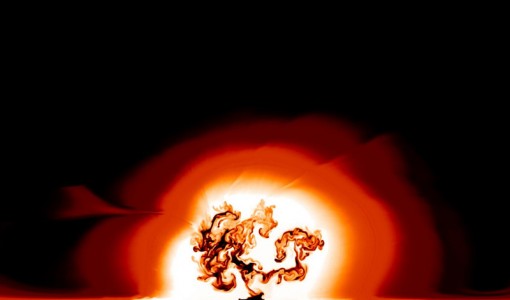
Image showing the asymmetric and turbulent flame that has consumed a white dwarf star in a type Ia supernova explosion. (Image credit: Daniel Kasen, UCSC)
Researchers use Jaguar to simulate ignition in type Ia supernova explosions.
Type Ia supernovas are the largest thermonuclear explosions in nature, expelling mass greater than that of the Sun and many of the basic elements of life. The long-standing mystery of these exploding stars lies in precisely how they explode.
Thanks to simulations on “Jaguar,” a Cray XT4 supercomputer at Oak Ridge National Laboratory (ORNL), a team of scientists has determined that Type Ia supernovas can explode asymmetrically and that this asymmetry would greatly affect their brightness.
“What we are trying to do specifically with this research is follow the evolution of the white dwarf from the first burning to supernova,” said principal investigator Stan Woosley, professor of astronomy and astrophysics at the University of California–Santa Cruz (UCSC). The other two members of the team are Daniel Kasen, also of UCSC, and Fritz Roepke of the Max Planck Institut in Garching, Germany. The team’s accomplishments were published in the August 13, 2009, edition of Nature.
Studying an exploding star has obvious complications. No laboratory on Earth could recreate an explosion of the caliber of a Type Ia supernova, nor could any human survive the resulting radiation. Moreover, researchers need computational tools that can scale down to the centimeters-thick flame that propagates through the star, all the way up to the turbulent explosion of a star. For the past 3 years, supercomputing resources at the National Center for Computational Sciences at ORNL have provided Woosley’s team with a machine powerful enough to run the codes necessary to study minuscule to stellar scales within such a supernova.
Life of a type Ia supernova
A Type Ia supernova begins as a white dwarf, a star of low to medium mass—similar to the mass of our sun—that has burned through most of its nuclear fuel and is no longer massive enough to generate fusion. These Type Ia precursors are composed mostly of carbon and oxygen, but also contain trace elements that affect the brightness of the explosion.
The best theorized mechanism by which white dwarves become Type Ia supernovas is within a binary, or two-star, system. In a binary system, the white dwarf pulls matter away from a companion star, making its way toward a mass that is about 40 percent greater than that of our sun, but with a radius one-fourth Earth’s.
The mass of a white dwarf cannot exceed this limit, referred to as the Chandrasekhar limit, without collapsing. Beyond the central density implied by this mass, even electrons moving at the speed of light cannot resist the huge gravity pulling the star inward. However, white dwarves that become Type Ia supernovas do not collapse as they still contain unburned nuclear fuel in the form of carbon and oxygen. As the companion star provides more mass, the white dwarf’s mass and density increase, causing the core temperature to build as a result of compression.
Burning carbon releases energy that drives convection. Like water at a rolling boil, plumes of hot, ionized gas called plasma rise from the center of the star to the surface, cooling as they rise. Convection continues for about a century, with carbon burning faster all the while until final, cataclysmic, runaway carbon fusion occurs at a temperature of 300 million degrees Celsius, lighting a nuclear “flame” that consumes the remaining carbon in the star.
“Most of the star burns in about a second once the explosion begins, creating about a solar mass of iron and maybe a couple tenths of a solar mass of other elements,” explained Woosley. The radioactive fallout created by the decay of nickel-56 to iron during the explosion releases enough energy to make the supernova as bright as several billion suns for a few weeks.
Simulating a supernova
Funded by the Scientific Discovery through Advanced Computing program of the U.S. Department of Energy’s Advanced Scientific Computing Research office, the current set of simulations by Woosley’s team began in 2007. Studies of Type Ia explosions by other groups often placed the ignition point at the spherical center of the star, but Woosley, Kasen, and Roepke questioned this assumption. The researchers’ first goal was to determine whether a central point of ignition could trigger a detonation capable of creating the explosions characteristic of Type Ia supernovas. MPA-FT, a software code developed by Roepke and collaborators, tracked the thin flame that consumes the star just before its explosion. This code created three-dimensional models showing that a central ignition point caused a rather weak explosion that did not produce many radioactive elements.
The next logical step was establishing how variations in the ignition geometry might affect the outcome of the explosion. Roepke produced numerous two-dimensional models, each model varying in the number of ignition points and the distance from these points to the center of the star. These models showed ignition occurring tens of kilometers off center, while the first detonation took place closer to the surface of the star, leading to an asymmetrical explosion and a bright supernova.
The light curves and specta for the models were calculated using a multidimensional Monte Carlo code called Sedona. A general Monte Carlo code is a computational algorithm that simulates physical and mathematical situations using repeated calculation of random samples. The multidimensional aspect of the code mimics the dimensionality of the explosion of a nonspherical object. Sedona, developed by Kasen, calculates the aftermath of the explosion.
“We use it to simulate different packets of light being generated from the radioactive debris of the explosion, and then we follow the trajectories of the light waves until they escape,” explained Kasen. “When we do this for tens of millions of these light packets, we can make a picture of what the debris of the explosion looks like. This is how we connect supercomputer simulation to observations of actual supernovas.”
Making a connection to documented observations of actual supernovas is the only way in which astrophysicists can validate their computer simulations. Spectra and light curves calculated from the two-dimensional models agreed with data collected from other well-observed Type Ia supernovas.
Type Ia supernovas have been invaluable to studies of the universe. Astronomers can calculate the distance to Type Ia supernovas because the maximum light emitted from the explosion is correlated with the time it takes to brighten and fade. Type Ia supernovas produce constant total light output because of the uniform size of carbon-oxygen white dwarves, and the near constant amount of nickel-56 produced in each explosion. A shift in the light emitted toward the red, less energetic end of the electromagnetic spectrum tells scientists that the supernova is receding. Observations of Type Ia supernovas have provided the strongest evidence of the existence of dark energy, a theoretical form of energy that pervades space, driving its expansion.
By studying first principles of how the supernova explodes, the team aims to refine distance calculations, strengthening the use of Type Ia supernovas as proof of the expansion of space.
Woosley and his team have shifted their sights to three-dimensional modeling of ignition. A code dubbed MAESTRO is currently running on Jaguar to provide even higher resolution simulations of the convective phase that occurs prior to ignition. MAESTRO tracks fluid motion in the presence of nuclear reactions and gravity over an extended amount of time. The researchers have applied for more time on Jaguar through the Department of Energy’s INCITE program to focus on other phases of the Type Ia supernova explosion.
The connection between Type Ia supernovas and the lives of humans on Earth may seem like a stretch, but understanding exactly how these stars explode helps scientists improve their theories on how mankind evolved.
“Two-thirds of the iron in our blood comes from these thermonuclear explosions from billions of years ago,” explained Woosley. “We are all the great-great grandchildren of supernovas.”
— by Caitlin Rockett