At the turn of the 20th century, scientists discovered that atoms were composed of smaller particles. They found that inside each atom, negatively charged electrons orbit a nucleus made of positively charged protons and neutral particles called neutrons. This discovery led to research into atomic nuclei and subatomic particles.
An understanding of these particles’ structures provides crucial insights about the forces that hold matter together and enables researchers to apply this knowledge to other scientific problems. Although electrons have been relatively straightforward to study, protons and neutrons have proved more challenging. Protons are used in medical treatments, scattering experiments, and fusion energy, but nuclear scientists have struggled to precisely measure their underlying structure—until now.
In a recent paper, a team led by Constantia Alexandrou at the University of Cyprus modeled the location of one of the subatomic particles inside a proton, using only the basic theory of the strong interactions that hold matter together rather than assuming these particles would act as they had in experiments. The researchers employed the 27-petaflop Cray XK7 Titan supercomputer at the Oak Ridge Leadership Computing Facility (OLCF) and a method called lattice quantum chromodynamics (QCD). The combination allowed them to map subatomic particles on a grid and calculate interactions with high accuracy and precision.
“Being able to perform these calculations and precisely quantify the interactions among the particles in a proton is essential to gaining a better understanding of the proton and a better understanding of lattice QCD as a whole,” Alexandrou said. “For example, if we find something new from these types of calculations that is not shown in experiment, we might need to reevaluate our theoretical concepts. That would be a significant finding, of course.”
Only a leadership-class system such as the OLCF’s Titan is capable of executing such heavy QCD calculations in a practical amount of time, the team said. The OLCF is a US Department of Energy (DOE) Office of Science User Facility located at DOE’s Oak Ridge National Laboratory.
“Titan was perfect for us because of its hybrid architecture,” Alexandrou said. “We would not have managed to do this calculation without this kind of capability.”
The feat is significant because modeling the proton structure from lattice QCD will provide important information about how matter is held together at the subatomic scale. A deeper understanding of QCD could also allow researchers to explore the nature of the early universe or even point toward new physics beyond current understanding.
A disappearing act
During a high-energy particle collision, an electron smashes into a proton, shaking up the proton’s fundamental components and then bouncing off. The proton is made up of three elementary particles—called quarks—as well as the gluon particles that act as carriers of the “strong force” that binds the quarks tightly together like a bag of marbles. The quarks—or “partons,” as they were originally termed in 1969 by physicist Richard Feynman—exchange momentum with the electron at the point of contact.
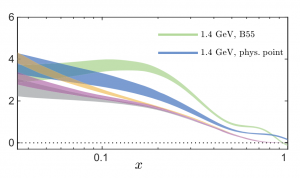
A comparison of an unpolarized parton distribution function using an ensemble from a phenomenological determination made from experiment (green) versus an ensemble from this team’s calculation on Titan (blue) at momentum ∼1.4 Gigaelectron volts. Image Credit: Constantia Alexandrou, University of Cyprus
When a quark gets “knocked out of the bag,” something interesting happens. Rather than revealing itself to the observer, the quark is immediately paired with an antiquark created from the vacuum of space, rendering the particle colorless, meaning it cannot be observed. Scientists, however, can use lattice QCD calculations to find out where the parton may be—and from where it may have come.
Lattice QCD allows for quarks to be positioned on grid points and gluons to be positioned on the links between these points. By using Monte Carlo statistical sampling methods, advanced algorithms, and large computers, scientists can accurately sample the QCD vacuum, the state at which matter has the lowest amount of energy. Supercomputing is essential to lattice QCD because the larger the grid is and the closer together the grid points are, the more accurate the simulations can be.
“We have this well-defined theory that says the proton isn’t an elementary particle,” Alexandrou said. “So we are trying to understand these partons and the overall proton structure—to determine what the proton is made of and what the properties of its constituents are.”
Using experimental data, scientists can deduce where a parton might be, but calculating its location from scratch proves more difficult because it requires massive high-performance computing resources.
The team, in collaboration with researchers at Deutsches Elektronen-Synchrotron-Zeuthen and Temple University, used lattice QCD and a method developed by Xiangdong Ji at the University of Maryland and Shanghai Jiao Tong University to identify the probable locations for a parton using only the underlying theoretical framework of the strong interactions—a capability that can help them understand more precisely what is inside a proton.
“Studying the properties of protons is difficult because you cannot break them up and study them,” Alexandrou said, explaining that the strong force binds quarks so tightly within a proton that scientists must study the interior interactions to gain new insights. “Any composite system in nature, up until now, we could break. But we can never, ever break the proton, so we have to study the particles inside it.”
Gaining momentum with supercomputing
The complexity of the problem meant the researchers had to perform a number of steps to reach an answer.
The first step was to accurately simulate the QCD vacuum. Using the SuperMUC computer in Germany, the group simulated the gluons, quarks, and antiquarks in a vacuum full of negative-energy particles known as the Dirac sea. The entire vacuum measured about 5 cubic femtometers (1 femtometer is 10-15 meters). For comparison, one femtometer is 300 billion times smaller than the width of a grain of salt.
Next, Aurora Scapellato, a Marie Sklodowska-Curie fellow at the University of Cyprus, performed calculations on Titan that showed what happens to a proton when an electron ejects energy to it. The problem is additionally complicated by the fact that the proton must have a large amount of momentum while it is being measured.
“In many other calculations, we can probe the properties of the proton while it is stationary,” Alexandrou said. “Here, we have to give it a lot of momentum. This can get complicated, because our errors increase the more momentum we give it.”
The team used a code called QUDA—or QCD on CUDA, a library for lattice QCD calculations on GPUs—to perform thousands of measurements over a 2-year allocation through the Innovative and Novel Computational Impact on Theory and Experiment program. Ten years ago, the number of calculations using previous architectures would have been limited to around a hundred within the same time frame.
“It’s incredible how many more calculations we are able to do with Titan,” Alexandrou said. “We need even more calculations before we can begin doing simulations that are more accurate than experiment. And the ultimate goal is to find out something we don’t yet know.”
The team has been running simulations on bigger lattices and hopes to take the project to the next level with even more momentum. A larger amount of momentum will provide more accuracy—but only if there is enough computation to properly control for errors. Performing these kinds of calculations could give scientists a comprehensive picture of the proton’s structure and interactions.
The method also has the potential to be applied to other particles.
“Eventually, these calculations are going to be useful for guiding experimentalists,” Alexandrou said. “If we have detailed information about the proton, we can tell experimentalists what to measure, what not to measure, where to look, and where not to look. And through this process, we may even discover something completely new.”
Related Publication: Constantia Alexandrou, Krzysztof Cichy, Martha Constantinou, Karl Jansen, Aurora Scapellato, and Fernanda Steffens. “Light-Cone Parton Distribution Functions from Lattice QCD.” Physical Review Letters 121 (2018): 112001, doi:10.1103/PhysRevLett.121.112001.
This work received funding from the European Union’s Horizon 2020 research and innovation program, DOE’s Office of Science, and the National Science Foundation.
ORNL is managed by UT-Battelle for the Department of Energy’s Office of Science, the single largest supporter of basic research in the physical sciences in the United States. DOE’s Office of Science is working to address some of the most pressing challenges of our time. For more information, please visit https://science.energy.gov.