Using the Titan supercomputer at the Oak Ridge Leadership Computing Facility (OLCF), a team of researchers has calculated a fundamental property of protons and neutrons, known as the nucleon axial coupling, with groundbreaking precision.
Led by André Walker-Loud of the US Department of Energy’s (DOE’s) Lawrence Berkeley National Laboratory, the project also used computing resources at DOE’s Lawrence Livermore National Laboratory. The OLCF is a DOE Office of Science User Facility located at DOE’s Oak Ridge National Laboratory (ORNL).
By applying lattice quantum chromodynamics (QCD)—a numerical method for calculating the underlying physics of the subatomic particles that make up protons and neutrons known as quarks and gluons—the team calculated the nucleon axial coupling with an unprecedented 1 percent precision, meaning all their computational results were in close agreement, or reside within a narrow distribution. The results also accurately matched long-standing experimental results.
“Lattice QCD is the only way we know how to compute properties of strongly interacting matter directly from fundamental theory,” Walker-Loud said. “For about a decade it has been an important predictive tool for high-energy physics.”
Although lattice QCD is often applied in the atom-smashing world of high-energy physics where scientists model particles interacting within very short distances, it has been a less manageable approach at the size of protons and neutrons (known as nucleons for their essential role in forming nuclei). Instead low-energy, or nuclear, physics problems are often modeled using data-driven, approximate methods.
The new nucleon axial coupling calculation, published in the journal Nature, provides the research community with a critical benchmark for applying lattice QCD to nuclear physics problems. Ultimately, this and other calculations enabled by the team’s computational technique could aid in the search for dark matter and help answer other outstanding questions about the nature of the universe.
Fundamental forces
Of the four fundamental forces by which matter interacts, the “strong” force gets its name for being stronger than the other three forces: the weak force, electromagnetism, and gravity.
Because the strong force is exerted at short distances—the space between particles in the nucleus of an atom—it is perhaps not as well known as gravity or electromagnetism, which can affect matter at visible scales. The strong force shapes an atom by bringing together, first, the quarks that make up nucleons and, second, the nucleons that make up the nucleus.
When scientists refer to the “underlying” or “fundamental” physics of nuclei, they are talking about the strong force interactions of quarks and gluons, including the property of color charge that influences how quarks are bound together and from which the study of quantum “chromo”-dynamics gets its name. Lattice QCD formulates these strong force interactions on a 4D grid, or lattice, representing space-time.
The weak force is also a lesser known but fundamentally important force in nuclear physics because it drives the decay and transformation of an unstable particle into other particles, contributing to the rich diversity of elements and their many properties.
By changing a down quark to an up quark, a weak force interaction known as the weak axial current drives the decay of a neutron into a proton. The nucleon axial coupling, or gA, is the strength by which the weak axial current couples to nucleons, which are held together by the strong force. Influenced by both of these fundamental forces, the nucleon axial coupling is an important value for making predictions about neutron decay and exploring new physics beyond the current Standard Model of Particle Physics.
“When we talk about the possibility of using lattice QCD for nuclear physics models, members of the experimental community often say ‘Come back when you have gA’ because it is such an important benchmark quantity,” Walker-Loud said.
Neutron decay is a current topic in dark matter searches, among other questions related to the nature of matter. If dark matter particles are released in neutron decay, as some theories suggest, then it might explain the presence of dark matter in the universe and offer a means of detection.
“There is a giant experimental effort to look for new physics in nuclear physics backgrounds,” Walker-Loud said. “Dark matter detection is one example. Other examples include looking for slight deviations from the predicted decay pattern of neutrons for which you have to be able to compute the contributions very precisely.”
The main reason it is difficult to apply lattice QCD problems to low-energy nuclear physics and get such precise computations, even as computing power increases, is known as the “signal-to-noise” problem. Just as large error bars clutter a graph, uncertainty or noise in computational results complicates researchers’ efforts to extract the signal they are seeking.
In the case of nuclear physics applications, a small subatomic particle known as the pion, which mediates nucleon interactions, introduces a lot of uncertainty in lattice QCD calculations.
“The tiny mass of the pion particles generates a lot of unwanted, statistical noise in the results,” Walker-Loud said.
Reducing the pion mass toward its physical value, which is tiny compared with the neutron mass, is important to accurate calculations but exacerbates the signal-to-noise problem.
Because QCD is a strongly interacting theory, creating an isolated neutron from the vacuum in lattice QCD calculations is nearly impossible. Instead, the neutron is simultaneously created with many other “excited” energy states, such as “breathing” or “resonating” modes of the neutron. As time progresses, these excited states decay away, leaving only the lowest energy state or “ground state” neutron. To calculate properties of the neutron, such as the nucleon axial coupling, these excited state contributions must be filtered out. Ironically, as the excited states decay away later, the signal-to-noise problem increases.
Using traditional lattice QCD methods, calculating the nucleon axial coupling can be a no-win situation.
Because of these and other computational challenges, the research community predicted that computing the nucleon axial coupling with a total uncertainty of 2 percent would take a couple more years and require next-generation supercomputers.
“For us to do it with 1 percent uncertainty required us to develop new ideas,” Walker-Loud said. “We have to continue to develop new strategies for computing that can accelerate the entire application of lattice QCD to nuclear physics.”
Turning down the noise
The team’s improved computational strategy reduced the amount of statistical sampling needed for an accurate answer, and GPUs accelerated the subsequent computations.
“We used about a factor of 10 fewer samples than previous projects,” Walker-Loud said. “The most computationally expensive calculations could only be performed on Titan, which enabled us to do our calculations about 100 times faster than we would have been able to do so otherwise.”
To reduce the signal-to-noise problem, the team’s improved method first averaged the interaction of the weak axial current across many points in time as a neutron decays to a proton, as opposed to selecting one interaction time as in previous methods. Second, the method filtered out excited state data, providing the team more control over uncertainty and enabling access to the signal earlier in the simulation time when the data is most precise.
“This was an intense two-and-a-half-year project that only came together because of the great team of people working on it,” Walker-Loud said.
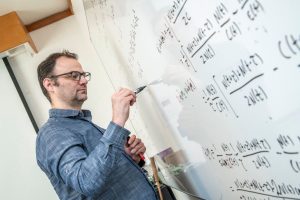
André Walker-Loud of Berkeley Lab led the computational study on the Titan supercomputer at OLCF. Image credit: Marilyn Chung/Berkeley Lab
The success of the project also relied on publicly available QCD configurations (which allow researchers to model how particles move on the lattice) from the MIMD Lattice Computation Collaboration; the lattice QCD code Chroma developed by USQCD; and QUDA, the lattice QCD library for NVIDIA GPU-accelerated compute nodes.
Walker-Loud said the data from Titan will allow the team to solve other physics problems by reducing the supercomputing cost of these additional results. The team also plans to pursue an even more precise calculation of the nucleon axial coupling on next-generation supercomputers, which could help resolve fine-grained discrepancies in existing experimental results and provide experimentalists looking for corrections to the Standard Model with more narrow search parameters.
This research was supported by the DOE Office of Science, including the Offices of Advanced Scientific Computing and Nuclear Physics, and by the National Science Foundation.
Authors include researchers and staff from Lawrence Berkeley National Laboratory; University of California, Berkeley; University of North Carolina; Brookhaven National Laboratory; Lawrence Livermore National Laboratory; Forschungszentrum Jülich; University of Liverpool; College of William & Mary; Rutgers, The State University of New Jersey; University of Washington; University of Glasgow; NVIDIA Corporation; and Thomas Jefferson National Accelerator Facility.
Related publication: C. C. Chang, A. N. Nicholson, E. Rinaldi, E. Berkowitz, N. Garron, D. A. Brantley, H. Monge-Camacho, C. Monahan, C. Bouchard, M. A. Clark, B. Joó, T. Kurth, K. Orginos, P. Vranas, and A. Walker-Loud, “A Percent-Level Determination of the Nucleon Axial Coupling from Quantum Chromodynamics.” Nature (2018), doi:10.1038/s41586-018-0161-8.
ORNL is managed by UT-Battelle for the Department of Energy’s Office of Science, the single largest supporter of basic research in the physical sciences in the United States. DOE’s Office of Science is working to address some of the most pressing challenges of our time. For more information, please visit https://science.energy.gov/.