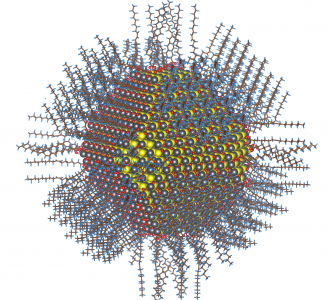
Complete atomistic model of the colloidal lead sulfide nanoparticle, also known as a quantum dot, passivated with oleic acid, oleyl amine and hydroxyl ligands.
Berkeley researchers use Titan to seek solutions to decades-old nanocrystal mysteries
Since their discovery over two decades ago, they have gone relatively unnoticed until recently. Now they are showing up in our TVs, smart phones, solar panels, and even our bodies. For something so small, we are beginning to see them everywhere.
Nanocrystals (NCs) are making a significant impact in materials sciences, resulting in better, more energy-efficient products, and in many cases at lower costs. This is because small is different; in other words, at nanoscale the relevant material properties and phenomena behave in interesting and useful ways—differently from how they behave at a scale, say, visible to the human eye.
The benefits of NC technologies are well known; however, on the atomic level, many of the characteristics and behaviors that make them so beneficial remain a mystery.
But thanks to the high-performance computing resources at the Oak Ridge Leadership Computing Facility (OLCF), they’re becoming less mysterious with the help of the Titan supercomputer, a Cray XK7 capable of 27 petaflops, or 27 quadrillion calculations per second. After completing a 3-year project, researchers from Lawrence Berkeley National Laboratory (LBNL) recently published two articles in the journal Science, revealing new insights into the NC atomic structure.
“Understanding more of the fundamental physics in different nanosystems, like how they grow, how the electrons behave, or how different molecules affect the system, allows us to utilize some control,” said lead investigator Lin-Wang Wang, a senior staff scientist at LBL. “And if we can control things like the crystal’s size and shape, we will be able to further advance their usefulness.”
The crystal soup concoction
When thinking about NCs, mind the scale—nanoscale is crazy small.
Picture a strand of hair: 1 nanometer is approximately 100,000 times smaller. Furthermore, each atom inside the crystals—arranged in a repeating, orderly 3D pattern—is 10 times smaller still. The structures Wang and his researchers deal with are typically about 5 nanometers, units with about 2,000 atoms each, called quantum dots (QDs).
It sounds intimidating, but surprisingly, synthesizing QDs is relatively simple; controlling their structure, however, is not.
“We call it a chemical cocktail,” Wang said. “Traditionally, laboratory experimentalists add ingredients like salt and acid [precursors] to organic solvents, or ‘soup,’ which, done under the right conditions, will cause the crystals to grow.”
Once that process is performed, within a matter of minutes a new crystal is formed. But saying “new crystal,” however, isn’t entirely accurate. NCs have the innate ability to regenerate. That is, if an NC is cut into smaller pieces, those smaller pieces will in fact grow back to their original shape during synthesis. Another way of describing it would be to say that once an NC reaches approximately 5 nanometers, growth in some cases abruptly stops.
But why do they do that? How do they do that? What’s happening on the NC surface that we can’t see? Are other molecules playing a role we don’t know about?
“At the atomic level, these were questions no one really had any answers to,” Wang said. “So the details of the atomic structure during passivation have for many, many years been like a black box.”
And according to him, a better understanding of what goes on during passivation is the key to opening that box.
“Imagine it like this,” said Danylo Zherebetskyy, a postdoctoral researcher in Wang’s group. “During passivation, organic molecules act like builders delivering bricks [atoms] to the particles’ surfaces. The molecules form extensions from the crystal’s center, almost like hairs that guide the atoms where to land, growing the crystal brick by brick.”
Those hairs are known as ligands, and not only are they responsible for growth, but they also influence how QDs interact with light, electrical charges, and other materials. And learning how to control ligand passivation, Zherebetskyy said, is critical in advancing nanotech applications.
Dissecting dots
Through an allocation from the OLCF’s Innovative and Novel Computational Impact on Theory and Experiment program, Wang and his team sought to answer those questions via a series of simulations, using Titan to calculate the surface energies and passivation patterns of lead sulfide and platinum nanocrystals.
Whereas laboratory experiments and various imaging methods could offer only hypotheses, for the first time Titan gave researchers precise predictions about NC surface development. Using the density functional theory codes VASP, LS3DF, and PEtot—quantum mechanics-based applications used to calculate the electronic and structural properties of molecules in many-body systems—researchers were able to develop an accurate, testable model, revealing an up-close examination of how each atom arranges itself within the system and how each of those molecules binds with other elements during passivation.
Because NC growth happens layer by layer, outward from the center, understanding the strange, abrupt stoppage in growth required the team to work from the outside in by slicing off sections of the NC surfaces. This process creates additional surfaces, each having different molecular arrangements and energies.
Wang explained: “For simplicity’s sake, let’s just look at two different surfaces. According to the old theory, based on principles in thermodynamics, the surface with the higher energy should grow the most because a higher energy means it’s more active, giving us an energetics picture. But what we find is just the opposite.”
As it turns out, the real secret to NC growth, Titan found, is ligand mobility.
“It takes a certain level of energy to displace a ligand on the surface, and that energy defines the ligand’s mobility,” Zherebetskyy said. “And the more energy it costs to displace the ligand, the more immobile it becomes.”
For growth to happen, he said, once a builder lays a brick, that ligand has to move out of the way so another atom can land next to it. Titan’s simulations made it clear, showing surfaces with lower energies facilitating the process, whereas higher energies actually create such a strong bond between the NC surface and the ligand molecules that passivation becomes completely blocked. Hence, NC growth abruptly stops.
“People have suspected this to be the case,” Wang said, “but until Titan, they never had the evidence to confirm that kinetic processes play a more important role than previously believed.”
The not-so-mysterious molecule
“I talk about size changing or controlling the properties, but the shape can also change the properties,” Wang said. “Different shapes will have different electronic structures and therefore will have different surfaces. Likewise, different surfaces require different methods of passivation.
“For almost 20 years we have been synthesizing inorganic [lead sulfide] quantum dots. But nevertheless, on the atomic level, no one has been able to figure out how the molecules attach to the particles’ surfaces.”
The reason, he explained, is that during passivation, a myriad of chemical reactions are taking place, making it impossible for physical testing or even advanced microscopy methods to identify every actor involved.
This was evidenced when the team placed a lead sulfide NC particle—recognized for its natural symmetry and its ability to form distinct facets—passivated with Oleic acid under Titan’s microscope to focus on unmasking unknown agents. By simulating the experiment, researchers can easily add or remove various molecules such as hydrogen and oxygen to observe their effects on passivation.
Consequently, because hydrogen and oxygen are two key ingredients of the Earth’s most valuable resource, it wasn’t entirely surprising when Titan revealed water to be the masked culprit behind the scenes of so many molecular mysteries. It was thought not possible because part of the synthesis process requires heating the precursor to 110°C (230°F), presumably eliminating any trace of water.
They found that water molecules occur as a byproduct of decomposition during crystal synthesis. Titan showed that water molecules, previously thought to exist only as a free-floating molecule within the soup, actually were a vital component in surface passivation.
“In the past, different experiments have revealed different features about surface passivation, from the amount of ligand molecules to the lead and sulfur atom ratios, but no one had ever put them together to agree with a single atomic model,” Wang said. “This is the first time all those observations actually fit.”
Truth be told
Titan’s findings subsequently aided the team in reproducing their experiments in the lab, giving them the physical confirmation needed to disprove several controversial theories.
The team produced large amounts of data by parallelizing their codes to take advantage of a significant number of Titan’s approximately 300,000 cores. Generated data sets were efficiently managed by routing them through the OLCF’s High Performance Storage System, a tape-based archiving tool.
“Without Titan our calculations would have taken forever, if not been outright impossible,” Zherebetskyy said.
“Our ability to simulate research has become a very powerful technique,” Wang added. “If you cannot do a simulation to get your result, then you probably still don’t fully understand your problem.”
Thanks to Titan and the many resources offered at the OLCF, a US Department of Energy Office of Science User Facility, the team was able to combine computations with various methods of physical testing to produce groundbreaking results in the advancement of new nanomaterials studies.
“I think these projects are just the beginning,” Wang said. “We now have a complete model. So that, I think, will open the door for future investigations into the surface states for more realistic calculations for the QD.”
—Jeremy Rumsey
Oak Ridge National Laboratory is supported by the US Department of Energy’s Office of Science. The single largest supporter of basic research in the physical sciences in the United States, the Office of Science is working to address some of the most pressing challenges of our time. For more information, please visit science.energy.gov.
Related publications:
Danylo Zherebetskyy, et al. “Hydroxylation of the Surface of PbS Nanocrystals Passivated with Oleic Acid,” Science 344, no. 6190 (2014): 1380–1384. doi:10.1126/science.1252727.
Hong-Gang Liao, et al. “Facet Development during Platinum Nanocube Growth,” Science 345, no. 6199 (2014): 916–919. doi:10.1126/science.1253149.